Regulation of focal adhesion assembly[Edit]
Factors regulating focal adhesion assembly, dynamics and function
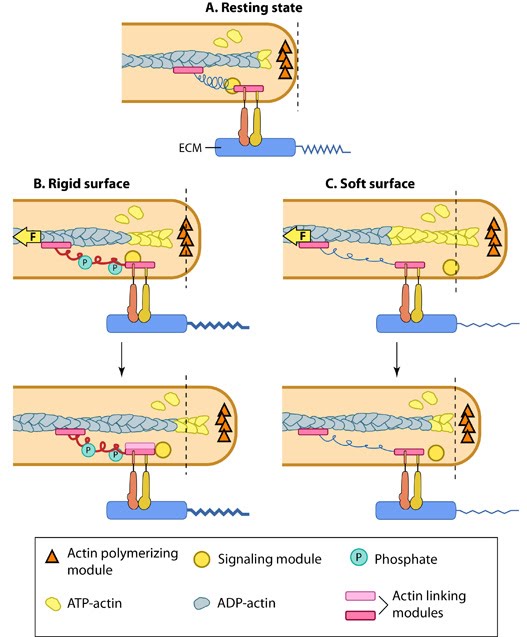
Substrate rigidity has a great impact on the morphology of certain cell types such as fibroblasts, but has no effect on neutrophils [12]. Rigidity sensing by focal adhesions can cause changes in FA dynamics and hence propagate signals that alter cell shape and cytoskeletal structures. For example, fibroblast polarization on rigid substrates is preceded by elongation, stabilization and uniform alignment of FAs along the elongation axis of the cell, whereas on compliant surfaces, small rounded and highly dynamic adhesions are formed and no proper polarization is observed [5].
Spacing of matrix ligands is also critical for establishment of stable adhesions. Cavalcanti-Adam et al. have shown that a spacing range of 50-70nm is required between ligand molecules, beyond which adhesions do not form and poor cell migration wase observed [13]. This distance seems to coincide with the length of talin, suggesting that talin is the principal mediator of integrin–actin linkage (reviewed in [14,15]). Independent of the global ligand density, it has been shown that nanoclustering of at least four integrin-binding sites in this threshold distance range is essential for effective integrin-mediated signaling [16]. Ligand spacing has also been demonstrated to be important for integrin signaling in endothelial cells [17].
2) Cycling of integrins is a key aspect of mechanotransduction by focal adhesions. Integrins get activated, cluster, segregate and disassemble throughout the different stages of adhesions. This process facilitates both the transition of early complexes to mature and the ability to rapidly disassemble. All these steps are force-sensitive and dependent on other adhesion components (reviewed in [18]) . Recently, it has been proven that integrins rapidly switch between active and inactive conformations within FAs [Rossier 2012]. This influences their trafficking and hence FA dynamics [Arjonen 2012].
3) Adhesion strengthening is required for nascent adhesions to survive shear forces exerted by actin retrograde flow. The friction between the adhesion components and hence the strengthening depends on the nature and organization of the receptor-ECM bonds (reviewed in [19, 20]), and is known to generally correlate with increasing ligand density [21] and the ability of integrins to cluster laterally [22].
However, the adhesive bonds for β1 integrins are stronger than that of β3 integrins [23, 24]. In β1 integrins, the adhesive bond is further enhanced by applied forces that increase the catch bond behavior of the molecule [25], altogether with the engagement of a synergy site in fibronectin [26]. Such catch bond behavior also fits a stochastic model that explains adhesion engagement state in relation to actin retrograde flow and corresponding traction stresses [27]. In β3 integrins, the adhesion strength is increased by linkage to actin [28] and sustained by force-dependent recruitment of components such as talin, vinculin [29, 30] and paxillin [31, 32], that reinforce the linkage [33] and initiate signaling cascades [34, 35]. Adhesion strength can modulate migratory behavior and shape in motile cells [36].
4) Flow of actin (i.e. actin retrograde flow)- The application of mechanical force that is generated by the actin system seems to be a prerequisite for the earliest stages of focal adhesion assembly and generation of traction forces [30, 31]. The coupling of the adhesion clutch to moving actin is mediated by the continuous association and dissociation of sliding molecular bonds referred to as ‘stick-slip behavior’ [37, 38].Quantification of traction stresses exerted on the ECM by FAs using high-resolution traction force microscopy revealed that intermediate actin flow rates correspond to maximal traction [39]. At the leading edge, where FAs form and flow is high, traction is minimal. However near the larger growing adhesion, the flow is slowed down [39, 40] to ~8-10nm/s and is linearly proportional to traction stress [35] (reviewed in [19]).
Two stochastic models explain this biphasic relationship of friction at the moving interface between flowing actin and stationary adhesions [27, 38]. The competition between the elastic bonds at the interface and energy dissipation in the viscoelastic actin interior is proposed to modulate the stability of adhesions [38]. The actin-receptor bonds are thought to reach a quasi-equilibrium state between bound and unbound forms. A catch bond model convincingly fits the experimental observations leading to clutch engagement and adhesion growth [27]. These also clarify why traction on the substrate is maximum at intermediate flow [40, 41].
5) Myosin contractile forces – FA growth, maturation and dynamics are highly dependent on myosin II contractility, that funnels the internal and applied forces to the adhesions as traction forces ([42, 43], reviewed in [44, 45, 46], see video below). MyosinIIA has been implicated in adhesion growth, remodeling of actin filaments into stress fibres and their maintenance between adhesions [47, 48, 49, 50] while Myosin-IIB is responsible for the formation of FAs at the rear and hence serve to establish cell polarity [51, 52]. However it is to be noted that mature adhesions can withstand about six folds increase in traction stress without any effect on their size [53].
During cell spreading, myosin contraction gets activated at early stages at the end of initial rapid spreading phase, when the cell transforms to a flat morphology, due to the physical barrier posed by the plasma membrane [54]. Thus increase in membrane tension seems to get converted into biochemical signal that activates actin assembly in the protruded area and causes local contraction that favors adhesion assembly [54]. In an independent in vitro study, Yu et al. have shown that upon early actin polymerization from liganded integrin clusters, myosin II contraction of clusters activates protrusion with the actin providing outward forces and the myosin providing the local contraction and inward movement [55]. This cyclic process known as the periodic lamellipodial contraction is mediated by myosin light chain kinase (MLCK) and the forces aid adhesion assembly and complete cell spreading [56]. Hence, one cannot ignore the possibility that the two mechanisms could be interconnected.